The Replacement Approach to Aging
Table of Contents
NOTE: This article is heavily inspired from the following presentation (opens in a new tab) by Dr. Jean Herbert. I'm by no means an expert on all the topics covered here. But, I wanted to create a resource for those who are new to the field of replacement. If you find any inaccuracies or have further insights, please feel free to message me on X (opens in a new tab).
Why Replacement?
Longevity has many treatment approaches, mainly: repair, rejuvenation, and replacement.
While repair and rejuvenation treatments are meaningful approaches, they cannot fully address physical, extracellular damage.
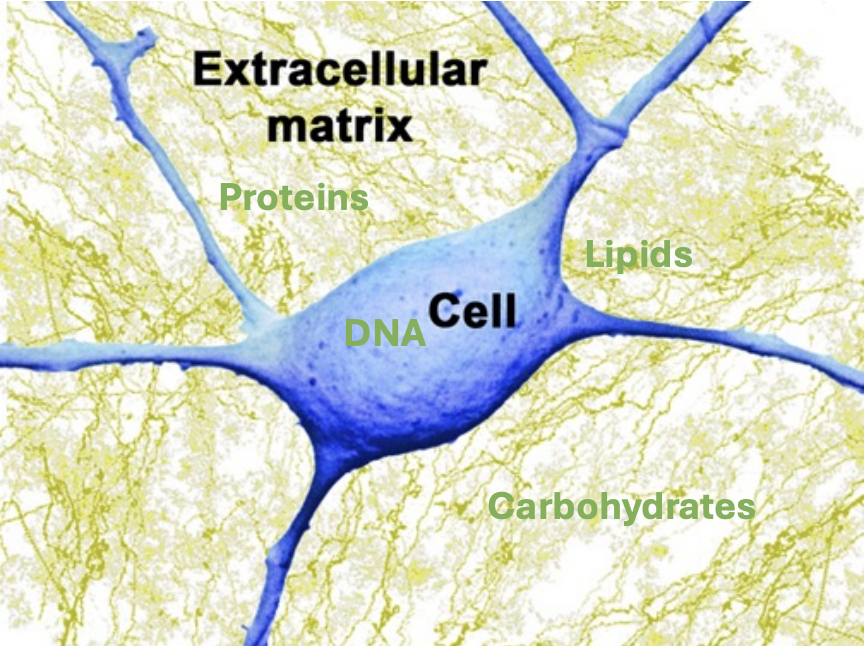
Millions of people die every year from diseases related to this type of damage:
Disease Category | Global Annual Deaths |
---|---|
Cardiovascular Diseases | ~18 million (WHO, 2019 (opens in a new tab)) |
Chronic Obstructive Pulmonary Disease (COPD) | ~3.5 million (WHO, 2021 (opens in a new tab)) |
Chronic Kidney Disease | ~1.2 million (NIH, 2015 (opens in a new tab)) |
Replacing your old body parts with new ones can prevent many of these deaths.
Also, much about aging remains unknown. We don't need to fully understand the science for the replacement approach to work, saving lots of time.
Finally, the transplant industry is already a multi-billion-dollar market with established talent and regulatory acceptance. Shifting its focus to aging would require less effort.
Replacement may offer the surest, fastest, and cheapest way to prevent aging.
Important Concepts
Grafts
TLDR: Moving tissue
A graft transplants tissue for repair. This is the crux of the replacement approach.
The first grafts attempted were for skin thousands of years ago in ancient India/Egypt. However, much of modern grafting started in the 1800s with skin graft techniques still used today (Kohlhauser et al., 2020 (opens in a new tab)).
These quickly developed into different types of grafts like bone, cartilage, and vascular (opens in a new tab).
While skin grafts, especially taken from a patient's own body (autografts (opens in a new tab)), are the most popular types of grafts today, the most useful for full body replacement are neural grafts. These began in the 1980s for Parkinson's (Boronat-García et al., 2017 (opens in a new tab)). For these, support structures (scaffolds) that mimic the brain's layout guide cells to form proper connections. These grafts try to replicate the layers of the brain. They provide spatial cues that help new cells align correctly and form proper connections with the host tissue. Early designs for these were basically simple hollow tubes that guided nerve growth (Gu et al., 2011 (opens in a new tab)). There have been many improvements in structure since then with physical fillers (Ruiter et al., 2009 (opens in a new tab)), multi-channel designs (Liu et al., 2021 (opens in a new tab)), and nanofiber architectures (Du et al., 2023 (opens in a new tab)). Now in clinical practice, single material grafts are the most common. Advancements in materials science engineering have created more hybrid, composite options, but these are still gaining popularity.
These guides have to balance the difficulties of integration. Support cells and growth factors are needed for this.
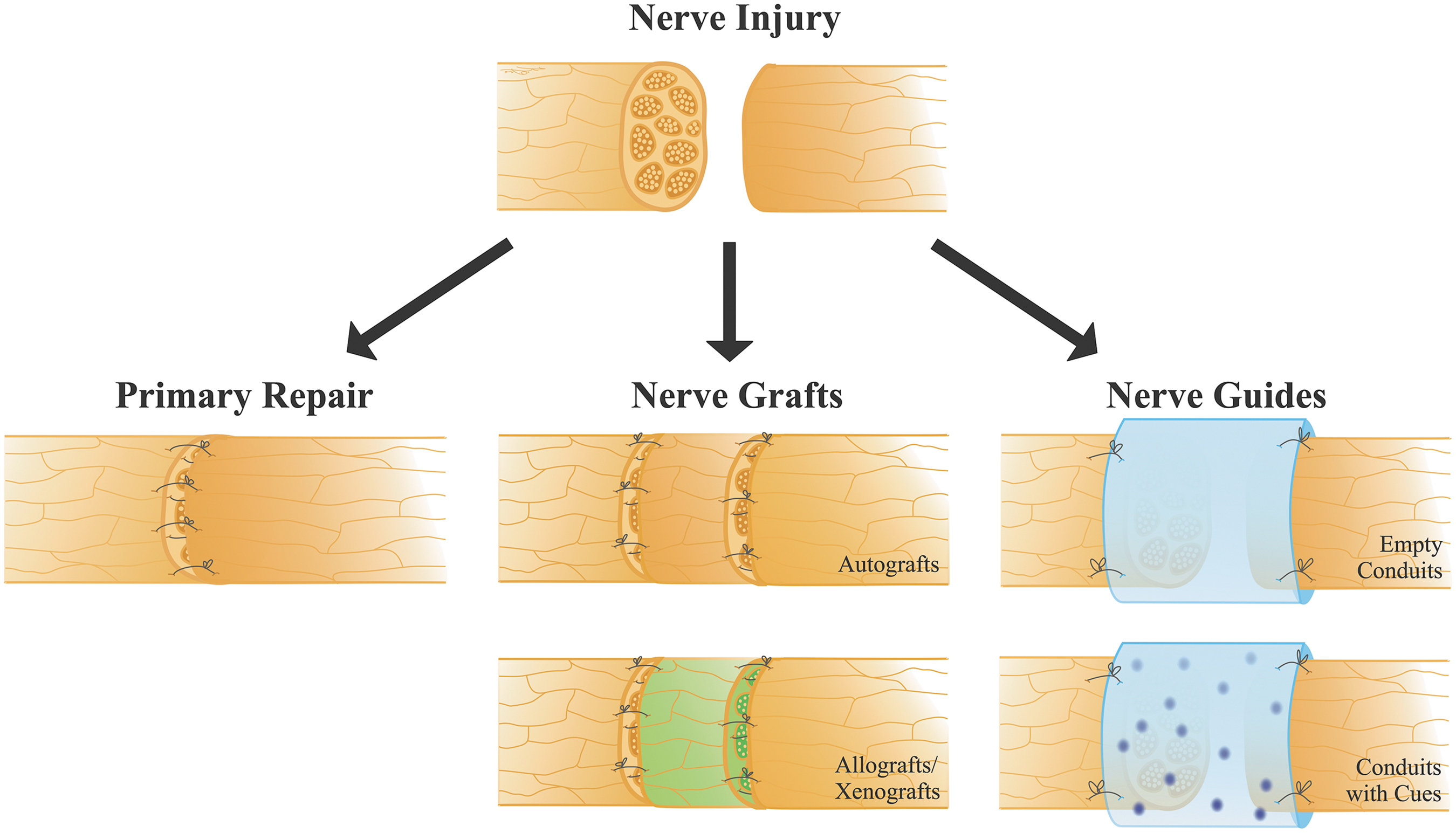
The perfect graft is a right mix of cell types (neurons, astrocytes (opens in a new tab), etc.) in the right 3D arrangement, ready to plug in and integrate with the host brain. New 4D bioprinting methods (opens in a new tab) could also let the scaffold adjust after implantation for a better fit.
We aren't there yet, but recent studies (Pasca et al., 2022 (opens in a new tab)) where human brain organoids were transplanted into rat brains and successfully connected (responding to sensory stimuli) show that substantial integration is possible. In the study, the organoids grew up, developing bigger cells and branch-like structures. They also reacted when exposed to air puffs and light. These reactions changed how the rats behaved, which could lead to new research directions.
Neural grafting is evolving from simply injecting cells and hoping for the best to carefully designing replacement tissue pieces that can fix damaged areas of the brain.
Immune Matching
TLDR: How can we align a donor's cells with the recipient's immune system?
Donor cells can be misidentified as foreign antigens by the recipient's immune system. This leads to a rejection of the cells, and the immune system attacks the transplanted tissue.
There are two popular solutions to this problem: immunomodulation and gene editing. Immunomodulation requires either immune drugs or retraining. Immunomodulatory drugs are given to the recipient to temporarily change immune signaling and detection. Retraining requires teaching the immune system to accept the new graft, often with specialized immune cells (T cells (opens in a new tab)). These solutions are usually temporary and generally weaken the immune system. In the short-term, they've been quite effective. Kidney transplants have had a 90-95% 1‑year graft survival rate for many decades (Pilch et al., 2020 (opens in a new tab)).
The newer, radical approach is gene editing. Donor cells are altered so the immune system has nothing to fight. This approach can last longer than immunomodulation. It nearly tripled the length of post-transplant survival for baboons (Eisenson et al., 2022 (opens in a new tab)).
These approaches are complementary. In the near term, gene-edited organs will still require some immunosuppression, as they aren't fully self-sustaining. The goal of easy, permanent tissue integration is still quite difficult and far away.
Vascularization
TLDR: Building networks of blood vessels to supply engineered tissues with oxygen and nutrients
Engineered tissues and large grafts need a blood supply. Without quick vascularization, transplants over a few millimeters in size die from lack of oxygen/nutrients within days. This development relies on two processes
- Angiogenesis - where new vessels originate from existing ones
- Vasculogenesis - where entirely new vessels form
Strategies to help with blood flow include adding pre-vascularized scaffolds (creating blood vessels in the lab instead), promoting growth factors (e.g. VEGF (opens in a new tab)), and selecting helpful materials. Scaffolds are usually made of biodegradable materials like collagen that degrade in weeks.
Vascularization is difficult because blood vessel lining cells (endothelial (opens in a new tab)) and the supporting cells are hard to source. Alterations to these cells often increase disease risk, so using a patient's own cells (autologous cells (opens in a new tab)) in living tissue (in vivo) has been increasingly studied. There has been some success in animal models for this (Brennan et al., 2008 (opens in a new tab)).
Becker et al. (2002) (opens in a new tab) offered a promising solution to this sourcing challenge through in situ (opens in a new tab) induced pluripotency (opens in a new tab) and dedifferentiation (opens in a new tab) of cells. Since then, we've gotten better at providing patient-specific vascular cells without the limitations of external sourcing (Sellahewa et. al, 2022 (opens in a new tab)).
The blood supply bottleneck might be solved with futuristic 3D-bioprinted capillaries, injectable vascular growth factor gels, or transplanted endothelial cell sheets (Sasmal et al., 2018 (opens in a new tab)).
Neuroplasticity
TLDR: The brain's ability to rewire itself allows new cells to integrate while preserving identity.
Plasticity preservation is about maintaining the brain's flexibility. It helps new cells form connections with pre-existing neural networks.
The two primary areas of plasticity are synaptic and structural. Synaptic plasticity changes the strength of neuron connections. It helps you quickly learn, remember, and make decisions every day. Structural plasticity alters the brain's wiring by growing new cells and dendrites. It supports long-term skills like forming habits.
The adult brain retains significant plasticity. Even in brain tumor conditions like glioma, plasticity allows the adult brain to adapt by rewiring functions to the unaffected areas (Taylor et al., 2023 (opens in a new tab)). This suggests that if small portions of aged brain tissue are incrementally replaced with new healthy neurons, the remaining brain can adapt to incorporate the grafts. It can relearn to use the new neurons with no sudden disruption of memory or identity.
Plasticity can be increased through molecular, physical, and electrical interventions. Molecular interventions use drugs, such as growth proteins and anti-inflammatory agents, to help neurons form new connections and stay healthy. Physical rehabilitation uses many techniques: physical therapy, targeted training, and even robot-assisted therapy to reinforce and reassign function. Electrical interventions use non-invasive brain stimulation to adjust brain activity and encourage neural reorganization (Zotey et al., 2023 (opens in a new tab)).
The goal is always for new tissue to preserve identity by not altering core functions.
Brain Machine Interfaces
TLDR: Devices that connect brain activity to machines to restore function
BMIs translate neural activity into commands for machines. You've probably already heard of examples like Neuralink. Current BMIs can restore some function for people with paralysis or sensory loss.
Both non-invasive techniques (like brain-to-text technology (opens in a new tab)) and minimally invasive approaches (such as blood vessel implants that help paralyzed people control screens (opens in a new tab) and ultra-small "neural dust" sensors (opens in a new tab)) are being tested.
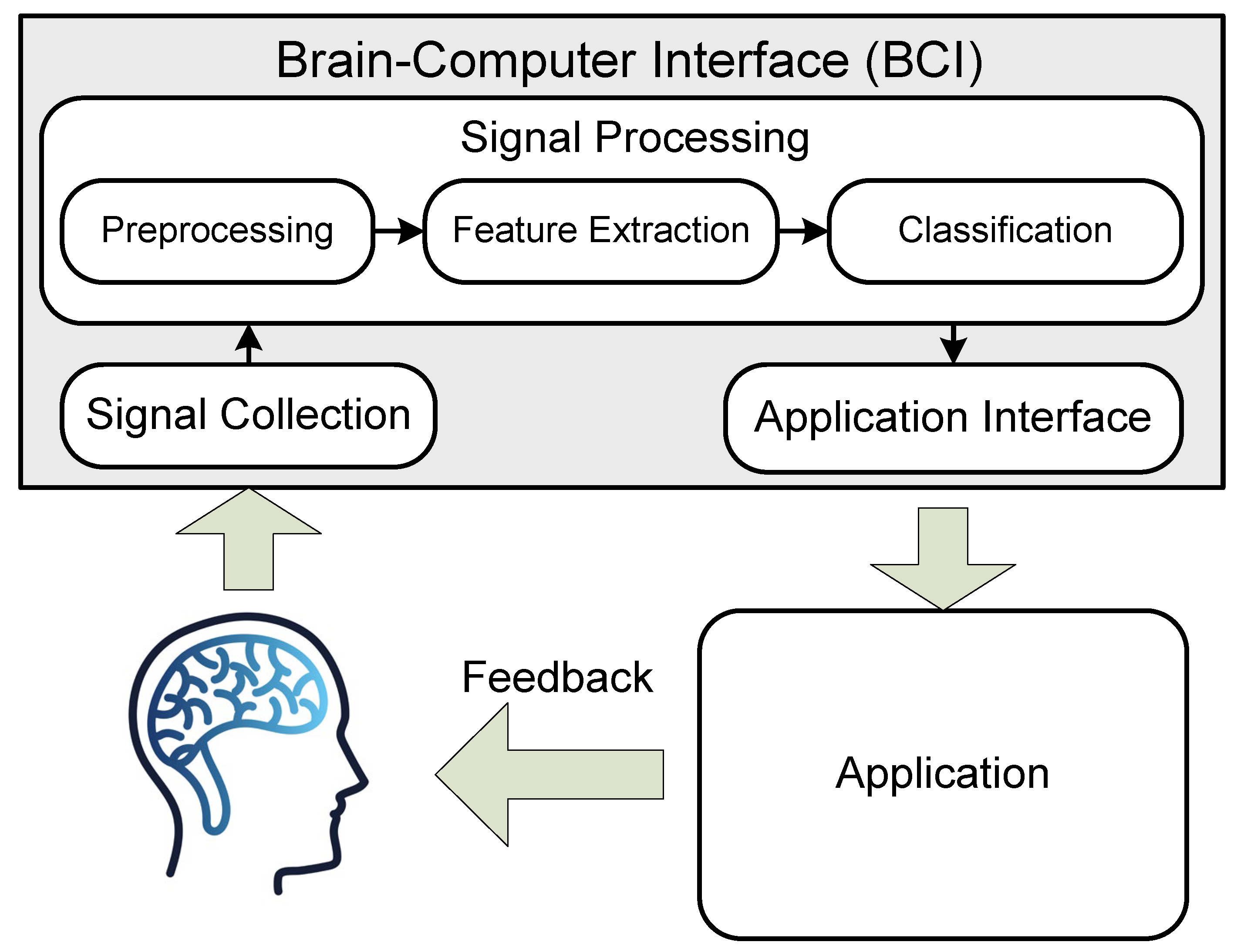
However, sending sensory information back to the brain remains a challenge. For example, a person controlling a robotic arm via BMI does not naturally feel what the arm touches. Most advanced BMIs today are invasive and can only replicate basic sensations. Making these systems both non-invasive and effective with prosthetics is particularly difficult (Voight et al., 2023 (opens in a new tab)).
BMIs could serve as a critical bridge for the replacement approach. Patients can use them to control devices while waiting for transplants or to restore lost brain functions. Moving forward, the biggest challenges for BMIs are invasiveness, bandwidth, and bidirectional communication, especially the "write" functionality that sends signals back to the brain.
Sentience Thresholds
TLDR: The theoretical point where consciousness emerges
Many bioethical issues arise in replacement. One major concern is determining whether transplant sources have consciousness.
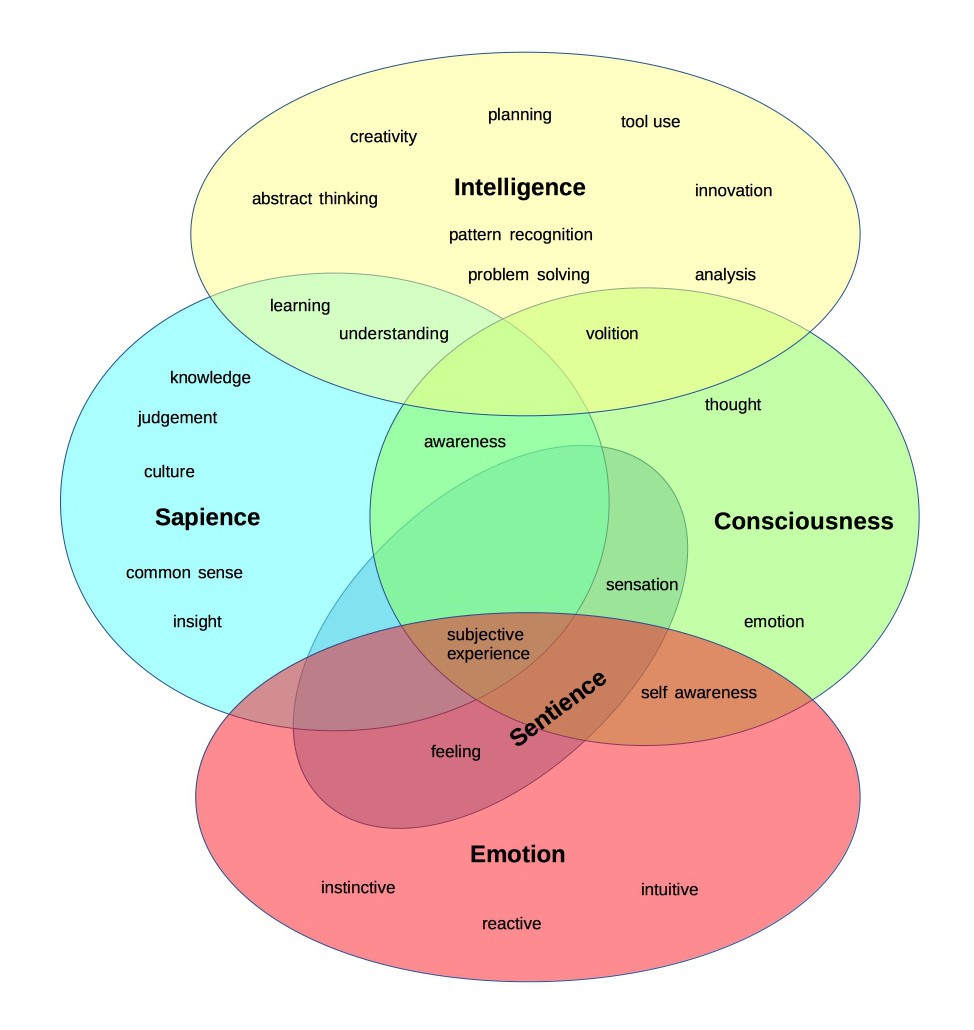
An interesting approach to solving this is decellularizing animal organs to create acellular (and thus "non‐sentient") scaffolds for use in preclinical studies. In this process, all the cells which carry the antigens that trigger immune rejection are removed from the donor organ, leaving behind just the extracellular matrix. This "body" has no life or consciousness and can be repopulated with the recipient's own cells. This technique has been around for a while (Ott et al., 2008 (opens in a new tab)) to generate a bioartificial heart scaffold from a pig heart.
Similarly, proposals exist to grow organs in animal models that lack a brain or consciousness. These could be anencephalic (opens in a new tab) human clones (if one were to be created) or animal chimeras (opens in a new tab) engineered so that any human neurons do not form a functional brain.
The closer a biological source is to sentience, the more problematic its use. Therefore, the best replacement strategies favor cells and tissues at pre-sentient stages. That could be embryonic or fetal tissue before a functional brain develops, or entirely artificial constructs that never had the capacity for consciousness.
(Note: There are definitely more concepts to add in this section. These are just what I think are most important.)
Implementation Strategies
Body Renewal Approaches
Biological
These methods let natural development do most of the work, without us having to fully understand every detail.
Whole-body
Pros | Cons |
---|---|
Everything (except brain) is young at once | Social resistance |
Potential for immune matching | Need to deal with severed spinal cord |
Requires little new tech | Scaling |
This strategy would give an aging person an entirely new, young body. This would be done by transplanting either their whole head or just their brain onto a donor body. This is the most straightforward replacement approach. Proof of concepts for many required technologies already exist, thanks to advances in brain-machine interfaces (BMIs) and biological regrowth. These donor bodies could potentially come from cloning. Scientists have successfully cloned primates, suggesting that similar techniques using human fetal cells might eventually enable human cloning as well.
One major challenge remains. We must bypass the severed spinal cord. Head transplants have been studied since the early 1900s with dogs (Lamba et al., 2018 (opens in a new tab)). They were even performed on monkeys who survived briefly (White et al., 1971 (opens in a new tab)). While these procedures showed promise, they raised serious ethical concerns.
No human head transplant has ever been done. Current related research focuses mainly on treating spinal injuries. Scientists are working to fuse damaged spinal cords. They're also developing BMIs to restore mobility (as described earlier). Solving either problem would remove the main technical barrier to head transplantation.
All Organs
Pros | Cons |
---|---|
No spinal cord severing | Social resistance |
Potential for immune matching | Some body parts remain old |
Requires little new tech | Scaling |
This approach is straightforward. It requires growing internal organs in a brainless body, then transferring them all at once to your original body.
The all-organs approach remains largely untested. Yet it could be simpler than expected. Most organs connect to the body in predictable ways. They typically have just a few major blood vessels, nerves, and specialized ducts entering and exiting. These limited connection points make surgical reattachment more manageable.
We could regenerate these organs using decellularization and recellularization approaches explained above. Work from other labs, like bladder reconstruction (Atala et al., 2011 (opens in a new tab)), shows that composite tissue systems with donor scaffolds and autologous cells could work.
Keeping original body parts like limbs is possible but risky. These remaining older tissues would continue to age, potentially leading to complications. For example, aged blood vessels are more prone to clotting, which could cause life-threatening pulmonary embolisms.
This method faces two major challenges: scaling up brainless body production and addressing ethical concerns similar to those of whole-body replacement.
Organ by Organ, Part by Part
Pros | Cons |
---|---|
No spinal cord severing | Many surgeries (greater risk of complications |
Scaling could be easier | Immune mismatch |
Already happening |
Instead of swapping the whole body at once, this approach requires bioengineering every major organ of an individual and replacing them one by one (or in groups).
This approach has several variations:
- Transplanting existing animal organs into a human (xenotransplant)
- There have already been procedures done in humans with pig organs (opens in a new tab). However, the immune rejection risk needs to be reduced for future success. The use of animals is not preferred, but in preclinical studies we have a way of making non-sentient bodies, as described earlier.
- Growing human organs in another species (interspecies chimeras (opens in a new tab)), then transplanting (blastocyst (opens in a new tab) complementation)
- Research by Kobayashi et al. (2010) (opens in a new tab) and Matsunari et al. (2013) (opens in a new tab) has demonstrated successful organ generation through interspecies chimeras. This process involves injecting human pluripotent stem cells into genetically modified animal embryos to grow predominantly human organs, such as a pancreas.
- Growing human organs in a lab (fetal tissue engineering)
- This approach has shown promise, with successful bladder engineering demonstrated in studies (Kajbafzadeh et al., 2018 (opens in a new tab)), though applications remain limited
Organ by organ replacement offers a more practical approach than whole-body solutions. There are existing techniques and each procedure can be specifically tailored to the patient.
Non-biological / Synthetic
Whole-body/All 'Organs'
Pros | Cons |
---|---|
Scaling | Social resistance |
BMI (brain machine interface) opportunities | BMI required |
More body parts remain old |
A non-biological whole-body replacement involves substituting all organs with synthetic alternatives.
Currently, only one project, BrainEx at Nenad Sestan's lab (Sestad et al., 2019 (opens in a new tab)), is working on complete whole-body organ replacement. Their goal is to develop a synthetic system that builds individual organs from advanced materials and links them with basic control interfaces.
Being engineered, this system could be maintained and upgraded similarly to a well-kept car, with any remaining biological parts potentially substituted by synthetic limbs.
Public acceptance of artificial organ systems will likely take time, as the concept is disruptive. BMIs would be required, particularly for controlling synthetic limbs. More research is needed to make this idea work.
Organ by Organ, Part by Part
Pros | Cons |
---|---|
Scaling | Many surgeries |
Bio-mismatch |
The organ-by-organ, part-by-part artificial replacement approach mirrors the biological process, but with man-made components.
Significant progress has been made in artificial organ development. Fully functional artificial hearts are already in use in patients (opens in a new tab). Additionally, kidney bioreactors (implantable devices containing kidney cells shielded by silicon-based immunoprotective membranes) have shown promising results in preclinical trials (opens in a new tab).
In most cases, the brain would remain biological, requiring biomachine compatibility, a significant challenge. While perfect compatibility remains elusive, advances have been made in blood vessel integration. Preventing detachment between biological and synthetic vessels is crucial, and the FDA has recently approved an important treatment advancement (opens in a new tab) in this area.
This organ-by-organ approach has clear, measurable progress compared to other methods. The technology appears achievable for most organs within the medium term.
Brain Renewal Approaches
Brain tissue replacement makes sense for two main reasons
- We can add new tissue that integrates:
- Research shows that transplanted embryonic precursors or other sources (like organoids or dissociated cells) can differentiate into neurons and integrate with the adult brain's circuitry. This means new brain tissue can be successfully introduced into existing networks. However, challenges remain, particularly in ensuring all necessary neuronal subtypes are present for proper information processing. Labs have repeatedly shown that transplanted embryonic precursors differentiate into neurons that integrate well into adult neurocircuitry (e.g. Goetz et al., 2016 (opens in a new tab)). It's not ready yet for fully functional neurons, as most studies are only for a single neuronal type. Without all neuronal types present, new tissue cannot process and organize information in a normal way. However, the proof of principle for the subparts of the overall solution is already present.
- We can gradually removal old tissue without functional loss:
- Clinical observations demonstrate that old brain tissue can be removed gradually without catastrophic loss of function or information. This has been observed in patients with slow-growing benign tumors, where abilities like speaking and personality remain intact. Because the tissue degrades slowly, the brain has time to reorganize and re-encode functions in different regions, avoiding the abrupt deficits seen with acute injuries like strokes. For example, patients with tumors developing in brain regions traditionally responsible for language maintained their ability to speak as these functions were reassigned to other areas (Gębska-Kośla et al., 2017 (opens in a new tab)).
The procedure would look like this
We have methods to progressively silence brain tissue, starting from a precise point and expanding outward. These methods aren't yet adapted to our specific purposes. These would mimic a glioma (as mentioned earlier). This would only require adaptations of existing technologies. It could be accomplished over the course of around 5-10 operations.
A few options for tissue replacement exist...
Engineering Tissue
Pros | Cons |
---|---|
Surgical implementation | Harder to get right |
Scaling |
This approach involves using tissue-engineered neural grafts (as discussed in the Grafts section) to replace small regions of an aging brain sequentially.
It requires surgical implantation. This approach enables easier scaling since you're designing the tissue and can adjust its unique shape to each lesion site.
However, engineered tissue replacement is more challenging than normal development, as you need to correctly identify the necessary extracellular signals and structural components
The Hébert Lab (opens in a new tab), for example, is tackling this by engineering fetal-like neocortical tissue based on omics analyses. They're identifying which precursor cell types and extracellular matrix signals are needed for proper development. Once implanted, these engineered cells mature into fully functional, structured tissue that can process information normally. Their animal studies are already showing promising results. A study from the lab (Quezada et al, 2023 (opens in a new tab)) supports these findings, indicating that neural tissue grafts from fetal tissue or iPSCs can mature and integrate after grafting. Testing larger grafts in stroke patients could be the next step to determine if they can restore lost cognitive function
An alternative is to grow neural tissue without any pre-formed connections ("blank" tissue). Unlike immature tissue that has basic synapses, truly asynaptic scaffolds lack any connections. This would allow doctors to program neural connections after implantation. The approach is still speculative. It could prevent random wiring and ensure precise integration with existing brain tissue, potentially speeding up recovery.
Engineered tissue is a painstaking but potentially definitive way to make an old brain young again.
Ex Utero 'Synthetic' Fetuses
Pros | Cons |
---|---|
Likely more authentic | Need for synthetic uterus for further growth and development |
Scaling |
The idea is to grow fetal brain tissue externally (outside a womb) that could be used for transplantation.
This could potentially be achieved using human pluripotent stem cells (hPSCs (opens in a new tab)), which can develop into various tissue types including neural tissue. We can now routinely culture hPSCs from individuals and differentiate them into early fetal-like tissue, essentially creating post-implantation human embryo models. The term 'synthetic fetus' is used because a developing fetal brain contains properly organized cell types and structures that would be more suitable for grafting than random organoids. Work from the Jacob Hanna Lab (Oldak et al., 2023 (opens in a new tab)) and the Magdalena Zernicka-Goetz Lab (Weatherbee et al., 2023 (opens in a new tab)) has shown that hPSCs can be coaxed into structures that mimic early post-implantation development.
These provide authentic, scalable models of early human tissue formation. However, these models haven't yet been matured to later fetal stages that would be ideal for graft collection. Without reaching these later stages, engineered tissues may suffer from necrosis, which is why a synthetic uterus-like environment would be necessary.
We likely wouldn't transplant a whole synthetic brain at once, but rather in stages like engineered tissue.
This entire category is very theoretical at the moment. It sounds appealing on the surface, but obvious moral issues of organ harvesting arise.
Interspecies Chimeras
Pros | Cons |
---|---|
Even more authentic | Scaling |
Xeno reactions |
Interspecies chimeras are organisms that contain cells from two different species.
In this context, the goal would be to grow human neural tissue in an animal host. However, as discussed, anything involving human neurons in animal brains is ethically fraught. We would not want to create a highly chimeric animal that might have a mix of human and animal cognition.
A more contained approach involves using animals as hosts for partial human brain development, similar to the synthetic fetus concept but within an animal (functioning as an in vivo bioreactor). However, this approach faces efficiency challenges due to potential immune reactions from the host animal, which would require immunosuppression
Instead of creating transplantable tissue, the real value lies in using chimeric models to study brain integration. Stanford's Pasca lab showed this by putting human brain organoids into rats to observe neural integration in a living brain (Pasca et al., 2022 (opens in a new tab)). They found human neural tissue matured far better in the rat brain than it ever did in lab dishes.
That implies that the in vivo environment is crucial. So one could foresee a strategy where a patient's iPSCs are used to grow organoids, those organoids are transplanted into a larger animal (like a pig) to "grow out" to a certain maturity (the pig might be engineered to accept them, e.g. an immunodeficient pig), and then after a period, that mature organoid is extracted and grafted into the patient's brain. This is convoluted (and you'd need to ensure no contamination with pig cells), but conceptually possible. It leverages the animal's body to do the nurturing of the tissue.
Interspecies chimeras have also been successfully demonstrated by introducing rat cells into mice. The Jun Wu Lab showed that rat stem cells injected into mouse blastocysts can contribute to various organs (Wu et al., 2017 (opens in a new tab)). Similarly, Kristin Baldwin's Lab demonstrated that rat neurons can restore the sense of smell in mice (Throesch et al., 2024 (opens in a new tab))
When successful, this approach beneficially relies on natural developmental processes rather than artificial engineering techniques.
Interspecies chimeras will likely play a supporting role in brain tissue replacement, as currently they are too high risk.
Human Fetal Tissue
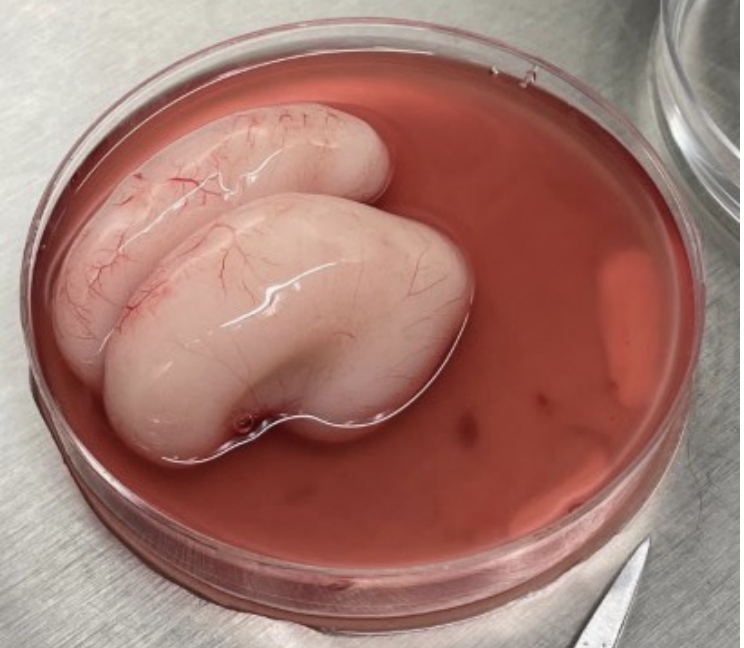
Pros | Cons |
---|---|
Authentic | Social resistance |
Scaling |
Human fetal tissue transplantation involves using immature neural cells from fetuses to replace damaged brain cells. Before stem cell technologies, the only source of young human neurons was fetal tissue from elective abortions.
It has been used since the 1980s to treat Parkinson's disease (as shown in research from Jiang et al., 1988 (opens in a new tab)). Very early embryos were transplanted into patients' brains to restore dopamine-producing cells.
Fetal grafts can work remarkably well for some patients but might cause side effects like abnormal movements (dyskinesias (opens in a new tab)) in others. This tempered enthusiasm for the procedure. Coupled with ethical controversy, especially in the US where federal funding was restricted, it led to a decline in fetal tissue use.
One transplant might require tissue from 3–5 fetuses to get enough cells. The need for pregnancies at precisely the right developmental stage makes this approach neither scalable nor controllable.
With advances in stem cell technology, the field has largely moved to using fetal-like cells derived in labs (so-called "fetal tissue replacement" without actual fetal tissue). These stem cells can create dopamine neurons that are more reliable and easier to produce (Barker et al., 2017 (opens in a new tab)). Because the cells are immature when transplanted, they don't come with full brain functionality. This helps ease some ethical concerns.
Fetal brain is the gold standard of plasticity and integration potential, but ethically and scalability questions remain.
Overall, brain renewal will probably involve combining methods. Perhaps lab-grown neural tissue will be supplemented by some ex vivo development trick and validated in chimera models, taking inspiration from fetal tissue studies.
Considerations
Scaling
Current transplant methods don't scale.
Organ stabilization, viability testing, and recipient matching need major updates. Thousands of organs are rejected every year due to insufficient time for testing and matching during the viability window immediately following the organ's excision from the donor (Cradle (opens in a new tab)).
Cryostasis is the largest potential solution to that problem, but only a handful of companies are working on it.
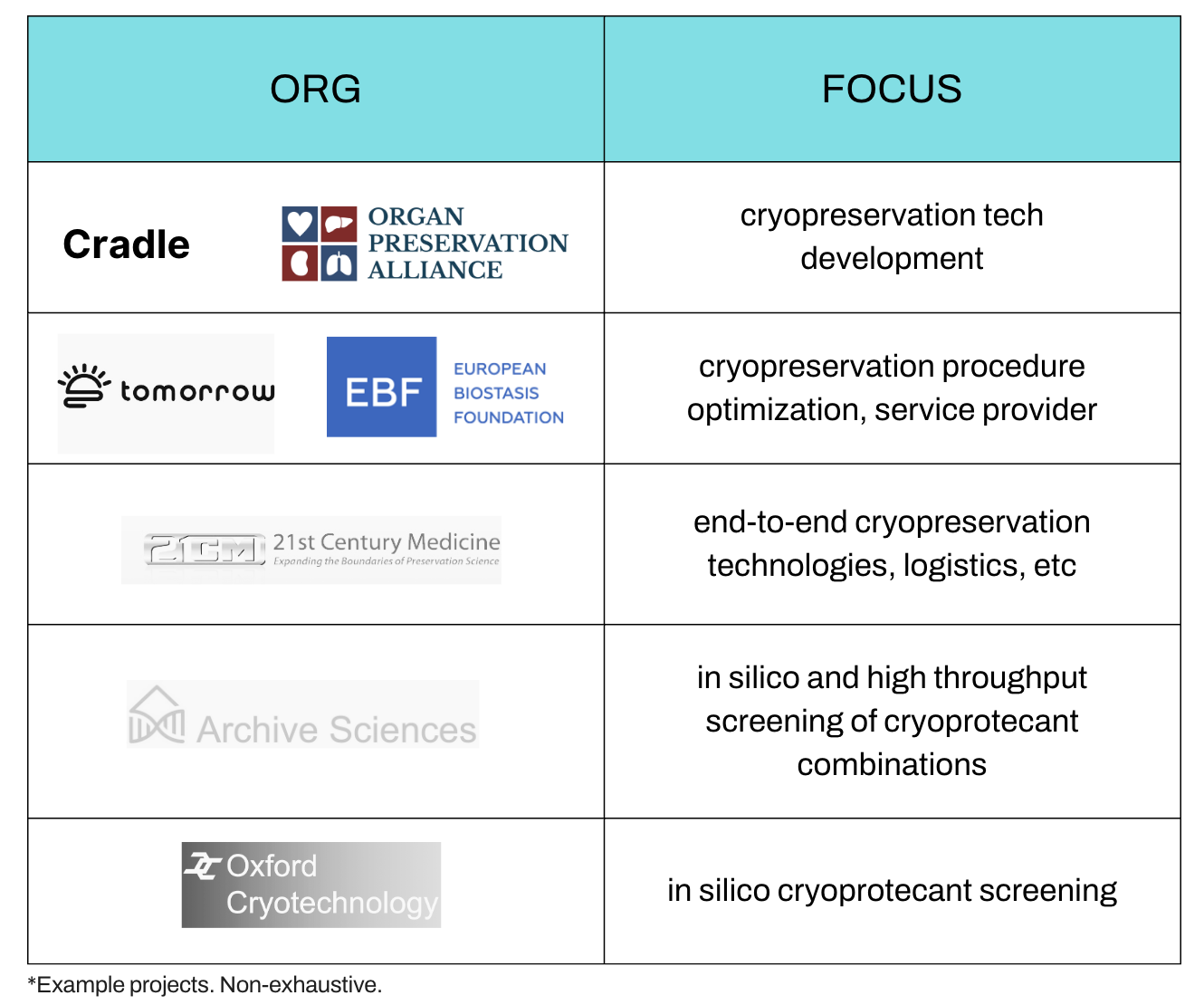
(Longevity Biotech Fellowship (opens in a new tab))
Availability
There is still a significant wait for most organs.
Organ Transplant Type | Median Waiting Time (2012-2021) |
---|---|
Heart | 190 days |
Heart and lung | 393 days |
Intestine | 255 days |
Kidney and pancreas | 650 days |
Liver | 433 days |
Lung | 79 days |
(Northwell (opens in a new tab))
Waiting times for most organs have decreased in the last 10 years, but not enough for rapid turnaround.
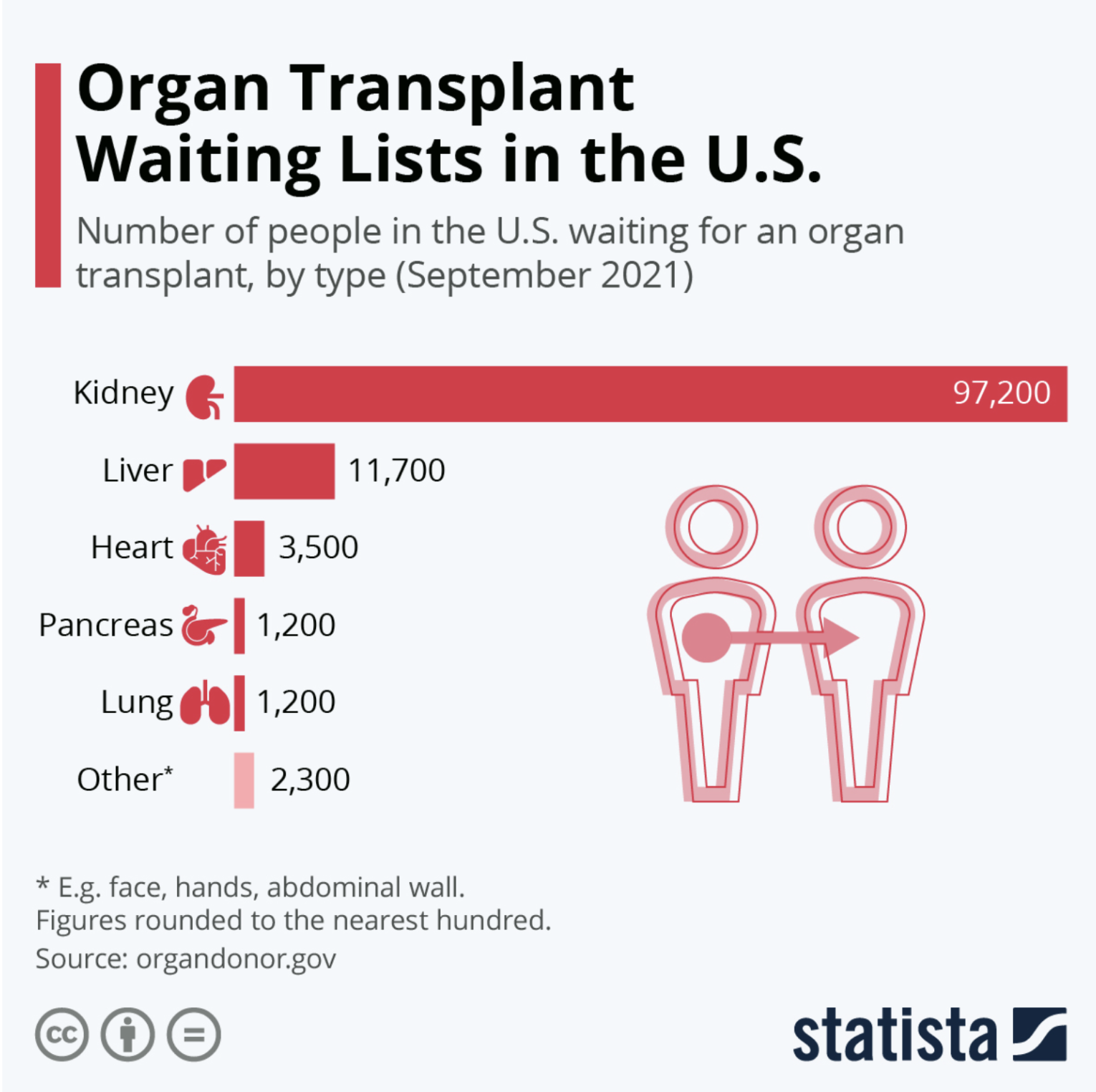
Waiting lists for transplants will continue to be necessary, especially as global health challenges such as rising obesity rates increase the demand for new organs
Accessibility
Transplants are high-tech procedures with limited global availability.
(Transplant Observatory (opens in a new tab))
A new alternative will have to be easily deliverable to many countries without existing medical infrastructure.
Pricing
Transplants are currently too expensive to be considered as a solution for aging.
This doesn't even account for the immunotherapy cost, sometimes up to $2,500 per month (James et al., 2015 (opens in a new tab)).
Legality
As mentioned earlier, one benefit of transplants is their relative lack of red tape. Transplants have existed for over 70 years, so there's been plenty of time to establish clear laws.
In FDA approval and in clinical settings, using individual cells and combinations of cells with scaffolds (though not complete tissues yet) doesn't seem to present regulatory obstacles.
The FDA appreciates and approves replacement strategies, even for the brain, because they work
Parkinson's:
- 1987: First dopaminergic neuron transplants into patients.
- 2022: Aspen Neuroscience raises approximately $250M total after Series B funding.
- 2023: Aspen Neuroscience receives Fast Track designation from FDA (their cells appear as effective as BlueRock's without requiring immune suppression because they are autologous).
- 2024: BlueRock Therapeutics demonstrates effectiveness in clinical trials.
Epilepsy:
- 2024: Neurona Therapeutics alleviates epilepsy in patients using iPSC-derived inhibitory neurons, with the company valued at $650M.
The current whole-body transplant market needs a major breakthrough to become scalable. Hopefully, advances in technology and economics will create economies of scale for the approaches described previously.
Development Timeline
An optimistic timeline of full body replacement from the Longevity Biotech Fellowship (opens in a new tab)
NOTE: Thank you to @theodorus5 (opens in a new tab) for reading a draft of this post.
RSS